Dynamical Drivers of the Exceptional Warmth Over Siberia During the Spring of 2020
The first half of 2020 was marked by unusual warmth over much of northern Asia (Collow et al. 2022). Of note was the persistence of warm anomalies over central Siberia from the winter into the spring, which contributed to devastating wildfires during June (Ciavarella et al. 2021). In a recent study (DeAngelis et al. 2023), we investigate the atmospheric dynamical drivers of the Siberian warmth during April and May. A key aspect of our approach is the use of atmospheric general circulation model (AGCM) simulations with prescribed atmospheric conditions over various regions (known as regional replay; Takacs et al. 2018; Table 1) to elucidate the remote regions that contributed to the event and the underlying physical mechanisms.

One clear factor contributing to the unusual Siberian warmth was the presence of quasi-stationary Rossby wave trains stretching from the North Atlantic to Eurasia in April and May, placing a persistent atmospheric ridge over central Siberia during both months. Figure 1 compares observed anomalies for April 2020 with results from the AGCM experiments. RPL_NA is the only experiment to reproduce the observed wave train and associated temperature anomaly pattern over Eurasia. This suggests the pronounced Rossby wave train that was strongly associated with the April temperature anomalies was forced from an upwind region over North America and the western North Atlantic. Supplemental analysis with a stationary wave model shows that dynamical forcing associated with enhanced storm activity over and downwind of the North Atlantic, likely related to internal atmospheric variability, was key to generating the wave train (DeAngelis et al. 2023). Similar results were also found for May 2020. This suggests the sub-seasonal predictability of the Rossby wave trains and associated Siberian temperature anomalies was likely limited.
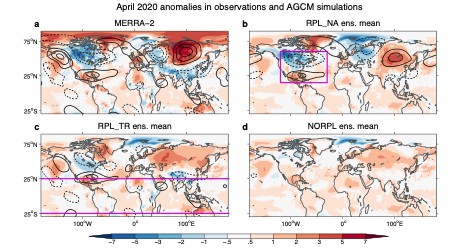
Despite not reproducing the April 2020 wave train, the RPL_TR and (to a lesser extent) NORPL ensembles nonetheless exhibit notable and relatively homogenous warm anomalies over Eurasia and other parts of the Northern Hemisphere (Figs. 1c,d). The fact that substantial warm anomalies appear over the Northern Hemisphere extratropics in RPL_TR suggests that a tropical-extratropical teleconnection process (apart from a wave train) also contributed to the warm anomalies over Eurasia.
The tropical-extratropical teleconnection can be traced to anomalously warm sea surface temperatures (SSTs) and associated upper-tropospheric heating in the tropics. Figure 2 depicts the teleconnection mechanism for April 2020 in the RPL_TR experiment (see DeAngelis et al. 2023 for a more comprehensive analysis). Specifically, the anomalously warm tropical upper troposphere strengthened the subtropical meridional temperature gradient (Fig. 2a). Through thermal wind balance, the strengthened atmospheric temperature gradient intensified the subtropical zonal jet stream at ~30N (Fig. 2b). The strengthened jet stream then altered the basic state through which atmospheric waves (or eddies) develop and propagate, causing anomalies in the convergence of horizontal eddy momentum flux in the mid-high latitudes of the upper troposphere (Fig. 2c). To satisfy the zonal momentum budget and atmospheric continuity, the Ferrel and Polar cells associated with the mean meridional circulation (MMC) slowed in response to the eddy momentum flux convergence anomalies (Fig. 2d). The slowed MMC ultimately led to adiabatic and diabatic heating anomalies, causing atmospheric warming between 45-75N (Fig. 2a). The mechanism outline above is consistent with the theory described in Seager et al. (2003) and is similar to what occurred during the previous winter (Schubert et al. 2022). That above average tropical SSTs (which are part of a long-term trend) played a substantial role in the teleconnection mechanism suggests long-term climate change may have contributed to the Siberian heat event.
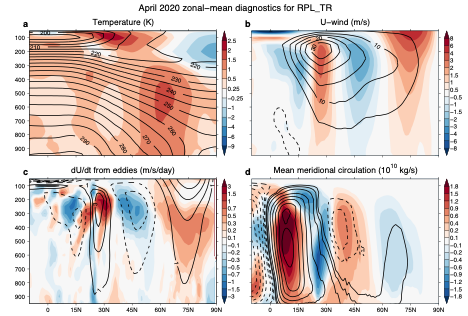
References
Ciavarella, A., and Coauthors, 2021: Prolonged Siberian heat of 2020 almost impossible without human influence. Climatic Change, 166, 9, https://doi.org/10.1007/s10584-021-03052-w.
Collow, A. B., N. P. Thomas, M. G. Bosilovich, Y.-K. Lim, S. D. Schubert, R. D. Koster, 2022: Seasonal variability in the mechanisms behind the 2020 Siberian heatwaves. J. Climate, 35, 3075–3090, https://doi.org/10.1175/JCLI-D-21-0432.1.
DeAngelis, A. M., S. D. Schubert, Y. Chang, Y.-K. Lim, R. D. Koster, H. Wang, and A. B. Marquardt Collow, 2023: Dynamical drivers of the exceptional warmth over Siberia during the spring of 2020. J. Climate, 36, 4837-4861, https://doi.org/10.1175/JCLI-D-22-0387.1.
Gelaro, R., and Coauthors, 2017: The Modern-Era Retrospective Analysis for Research and Applications, version 2 (MERRA-2). J. Climate, 30, 5419–5454, https://doi.org/10.1175/JCLI-D-16-0758.1.
Molod, A., L. Takacs, M. Suarez, and J. Bacmeister, 2015: Development of the GEOS-5 atmospheric general circulation model: Evolution from MERRA to MERRA2. Geosci. Model Dev., 8, 1339–1356, https://doi.org/10.5194/gmd-8-1339-2015.
Schubert, S. D., Y. Chang, A. M. DeAngelis, R. D. Koster, Y.-K. Lim, and Hailan Wang, 2022: Exceptional warmth in the northern hemisphere during January through March of 2020: The roles of unforced and forced modes of atmospheric variability. J. Climate, 35, 2565-2584, https://doi.org/10.1175/JCLI-D-21-0291.1.
Seager, R., N. Harnik, Y. Kushnir, W. Robinson, and J. Miller, 2003: Mechanisms of hemispherically symmetric climate variability. J. Climate, 16, 2960-2978, https://doi.org/10.1175/1520-0442(2003)016<2960:MOHSCV>2.0.CO;2.
Takacs, L. L., M. J. Suárez, and R. Todling, 2018: The stability of incremental analysis update. Mon. Wea. Rev., 146, 3259–3275, https://doi.org/10.1175/MWR-D-18-0117.1.